THE FEET FREE MOMENT AND GROUND REACTION FORCE IN WHEELCHAIR TRANSFER: A PILOT STUDY
Chung-Ying Tsai, MS1,2, Padmaja Kankipati, MS1,2, Claire Hoelmer1,3, Alicia M. Koontz, PhD, RET1,2
1Human Engineering Research Laboratories, Department of Veterans Affairs, Pittsburgh, PA
2Department of Rehabilitation Science and Technology, University of Pittsburgh, Pittsburgh, PA 15261
3Department of Bioengineering, University of Pittsburgh, Pittsburgh, PA 15261
INTRODUCTION
For wheelchair users, transfer skills are essential criteria for independent living because they are required for performing many daily activities. Wheelchair users need to transfer to and from their wheelchair to and from a bed, a commode, or motor vehicle every day. A full-time wheelchair user usually performs 14 to 18 wheelchair transfers per day (Finley, McQuade, & Rodgers, 2005) . The repetitive movements and internal joint loading associated with transfers are believed to lead to secondary upper limb pain and injuries (Gagnon, Nadeau, Noreau, Dehail, & Piotte, 2008). In addition, transfers carry an increased risk of falls and fall-related injuries and deaths (Saverino, Benevolo, Ottonello, Zsirai, & Sessarego, 2006) .
Persons with spinal cord injury (SCI) experience severe bone loss which causes osteoporosis in their paralyzed limbs over time (Chantraine, Nusgens, & Lapiere, 1986; Wilmet, Ismail, Heilporn, Welraeds, & Bergmann, 1995) . Low bone density causes the limbs to become more fragile and prone to fractures after only one to five years post-SCI (Lazo et al., 2001; Szollar, Martin, Sartoris, Parthemore, & Deftos, 1998) . Lower limb fractures are common injuries in people with SCI (Patatoukas et al., 2011). More than half (62.5%) of these fractures are below the knee, especially distal tibia and fibula (Lazo, et al., 2001). Because of the reduced ability of the paralyzed leg to withstand weight-bearing forces, even low-impact forces can cause fracture (Vestergaard, Krogh, Rejnmark, & Mosekilde, 1998). Transfers have been identified as one of the major activities linked to fractures (Fattal et al., 2011) yet most of the literature on transfers has been focused on upper limb biomechanics and overuse injuries (Gagnon, Nadeau, Noreau, Dehail, & Piotte, 2008; Gagnon, Nadeau, Noreau, Eng, & Gravel, 2008) . In order to prevent lower-limb fractures, a better understanding of the biomechanical risk factors is needed.
The feet free moment (FFM) is a moment caused by the friction force between the foot and the ground and acts along a vertical axis (Milner, Davis, & Hamill, 2006). A higher free moment is indicative of a higher torque acting on the tibia and ankle joint. Ground reaction force (GRF) and vertical loading rate (VLR) have also been used to analyze the causes of bone and stress fractures in several studies (Zadpoor & Nikooyan, 2011). GRF is the magnitude of vertical and horizontal forces imposed on the lower limbs by the ground. The VLR indicates the rate at which the vertical force is imparted to the lower limbs. These are considered important biomechanical variables for studying fractures in studies involving running, gait, and jumping (Caulfield & Garrett, 2004; Gottschall & Kram, 2005; Kiss, 2010).
The purpose of this study was to use these variables to study fracture risk for self-selected sitting-pivot wheelchair transfers (SS) and three prescribed sitting-pivot transfer techniques: 1) head-hip relationship with an abducted leading arm away from the body (HH-A); 2) head-hip relationship with an internally rotated leading arm close to the body (HH-I), and 3) with the trunk upright with an abducted leading arm away from the body (TU) (Koontz, Kankipati, Lin, Cooper, & Boninger, 2011a). These techniques were derived from the Clinical Practice Guidelines related to best transfer practices (McClure, Boninger, Ozawa, & Koontz, 2011; "Preservation of upper limb function following spinal cord injury: a clinical practice guideline for health-care professionals," 2005) and we questioned if fracture risk was dependent on type of technique.
METHODS
Subjects
The study was approved by the Department of Veterans Affairs Institutional Review Board. The inclusion criteria of the study were: 1) SCI at C4 level or below for at least one-year; 2) over 18 years old; 3) no upper extremity pain which would influence their transfers; 4) able to perform an independent transfer without any human assistance or assistive device. Eleven male subjects volunteered to participate in the study.
Experimental protocol
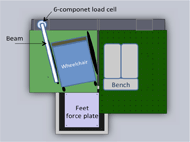
After informed consent, the subjects positioned their wheelchairs close to an adjustable bench on our transfer station (Figure 1) (Koontz, Lin, Kankipati, Boninger, & Cooper, 2011). The transfer station includes three force plates (Bertec Corporation, Columbus, OH) which are underneath the wheelchair, the bench, and the subject’s feet respectively, and one 6-component load cell (Model MC5 from AMTI, Watertown, MA) which is attached to a steel beam used to simulate a armrest. The bench was adjusted to a height level with the subjects’ own wheelchair seat. The bench and the subjects’ wheelchairs were secured to the aluminum platforms which cover the force plates.
All the subjects started the transfers with their left arm leading (hand placed on the bench) and right arm trailing (hand on placed the beam). For the self-selected transfer performed first by each subject, subjects were instructed to place their feet on the force plate, their left hand anywhere on the bench and their right hand anywhere on the beam. Subjects were asked to transfer to the bench as they normally would and then transfer back to their wheelchair. Three prescribed transfers (HHA, HHI, and TU)(Koontz, Kankipati, et al., 2011a) were taught to the subjects via standardized video-based instruction. For the three prescribed transfers, targets for their (left) leading hand and buttocks were placed on the bench to aid with executing each technique correctly. The prescribed transfer approaches were randomly assigned. Subjects were permitted time to practice each technique until they felt they could perform it well. Five trials were collected for each type of transfer with rest periods in between transfers. Kinetic data from all the force plates and load cell were collected at 360 Hz for the duration of each transfer.
Data analysis
The FFM can be calculated by combining the moment along the z axis and the moment caused by shear force (equation 1). Because the positive vertical axis of the force plate used in the study is downward, the negative FFM is the adduction free moment and positive FFM is the abduction free moment. The vertical and horizontal ground reaction forces (VGRF and HGRF), as well as vertical loading rate (VLR), are also important fracture risk variables. HGRF is the vector sum of the two horizontal components of GRF (equation 2). VLR is the slope between the initial VGRF and the peak of the VGRF. It indicates how fast the VGRF rise to the peak.
FFM = Mz - (CPx * Fy) + (CPy * Fx) (1)
HGRF = (Fx2 + Fy
2)
1/2 (2)
Equation 1 and 2: Mz is the moment along the Z axis. CPx is the x-coordinate of the center of the pressure. Fy is the y component of the ground reaction force. CPy is the y-coordinate of the center of the pressure. Fx is the x component of the ground reaction force.
A zero-lag low-pass Butterworth 4th order filter with a cut-off frequency of 7 Hz was used to filter the data. The vertical reaction forces from the load cell and the force plate underneath the bench were used to decide the beginning and the end of transfers. The transfer began when the load cell detected the hand force (rising from zero) (Kankipati, Koontz, Vega, & Lin, 2011). The end of the transfer was the moment before the landing spike of the buttocks was detected by the bench side force plate (Kankipati, et al., 2011). The dependent variables were the maximum, minimum, and average FFM (max_FFM, min_FFM, and ave_FFM), average and maximum of the vertical and horizontal components of GRF (ave_VGRF, max_VGRF, ave_HGRF, and max_HGRF), as well as VLR during the transfers. The FFM variables were normalized by the subjects’ height and weight and the GRF and VLR variables were normalized by subject body weight (BW). These variables were computed by Matlab (Mathworks, Inc., Natick, MA, USA) and averaged values were determined over the five trials of each transfer approach.
Descriptive statistics (means and standard deviations) were calculated and reported for each variable. However, because of the small sample size, the non-parametric Friedman’s test was used to compare differences in the fracture risk variables between the four transfer approaches with a level of significance at p < 0.05. All the statistical analyses were performed in SPSS (SPSS Inc., Chicago, IL).
RESULTS
The group mean (±standard deviation) of age, height, and weight were 37.6 (±8.9) years, 1.79 (±0.07) meters, and 74.91 (±17.21) kilograms, respectively. Their SCI level ranged from C5 to L1 with three having complete injuries and eight with an incomplete SCI. The average duration (±standard deviation) of post-SCI was 13.7 (±6.83) years.
The type of transfer appeared to have little influence on the fracture-risk variables (p > 0.1). However, there was a trend (p < 0.1) for the maximum FFM for the SS and HHA techniques to be higher than the maximum FFM for the TU and HHI techniques and for the HHA to have the highest maximum VGRF (Table 1). The adduction FFM (Min FFM) is about twice of the abduction FFM (Max FFM).
SS |
TU |
HHA |
HHI |
p |
|
---|---|---|---|---|---|
Ave FFM |
4.29 (±4.03) | 3.87 (±3.29) | 3.86 (±3.50) | 3.99 (±3.32) | 0.53 |
Max FFM |
5.47 (±5.06) | 4.44 (±4.63) | 5.41 (±4.83) | 5.05 (±4.29) | 0.07 |
Min FFM |
-9.63 (±9.31) | -10.54 (±8.80) | -9.42 (±8.07) | -8.89 (±7.95) | 0.45 |
Ave VGRF |
0.19 (±0.08) | 0.19 (±0.06) | 0.20 (±0.06) | 0.18 (±0.07) | 0.53 |
Max VGRF |
0.29 (±0.14) | 0.30 (±0.12) | 0.31 (±0.13) | 0.29 (±0.13) | 0.09 |
Ave HGRF |
0.04 (±0.03) | 0.03 (±0.02) | 0.03 (±0.02) | 0.03 (±0.03) | 0.71 |
Max HGRF |
0.07 (±0.06) | 0.08 (±0.05) | 0.08 (±0.05) | 0.07 (±0.05) | 0.66 |
VLR |
0.25 (±0.23) | 0.23 (±0.21) | 0.21 (±0.19) | 0.18 (±0.13) | 0.66 |
Note: Units, FFM(×10-3); VGRF and HGRF (%BW) ; VLR (%BW/s) |
DISCUSSION
Fractures resulting from transfers are largely under-researched and an attempt was made in this study to better understand the relationship between transfers and stress-related fractures of the lower extremity. The FFM has been considered one of the most important predictors for stress fracture in runners (Milner, et al., 2006; Pohl, Mullineaux, Milner, Hamill, & Davis, 2008) . If a runner has increased FFM in running, it is more likely for a stress fracture to occur. In these studies, the peak absolute FFM during running at 3.7 ms-1 was around 6×10-3 to 9×10-3 (Milner, et al., 2006; Pohl, et al., 2008) . The peak absolute FFM in the current study was about 10×10-3 which is very similar to the previous running studies. During sitting-pivot transfers, a person will typically orient their wheelchairs near the transfer surface at a 30 to 45 degree angle to get close to the surface and clear their buttocks of the rear wheel when performing the transfer (McClure, et al., 2011). As the FFM is the torque acting between the feet and the ground, this rotation during transfers is what produces a large FFM similar to that experienced in walking or running tasks. This combined with the weakened ability of the legs to withstand torsional loading due to bone loss post-SCI likely predisposes individuals who independently transfer to stress-related fractures. A stress fracture is a partial or complete fracture attributed to heavy repetitive loading. Joint loading of this nature may not result in fracture during an actual transfer but the micro-trauma that results from repetitive loading (e.g. 12-14 times per day) weakens the bone and eventually may lead to a fracture (Zadpoor & Nikooyan, 2011).
The peak adduction FFM (negative FFM) was larger than the abduction FFM (positive FFM). This is because the transfer direction in the study was from right to left (rotation to the right, right toes out). The shear and the torque between the feet and ground produced an adduction free moment to resist the rotation direction. The imbalance in ab/adduction free moments underscores the Clinical Practice Guideline recommendation to alternate direction of transfer whenever possible to avoid overuse on one of the upper limbs, and this appears to apply to the lower extremity as well. ("Preservation of upper limb function following spinal cord injury: a clinical practice guideline for health-care professionals," 2005).
The GRF has also been used extensively to analyze reasons for stress fractures in running. Higher vertical GRFs were related to runners’ tibia stress fractures (Zadpoor & Nikooyan, 2011). The values of the peak GRFs in these studies were around 2.4 to 2.8 BW. The maximum of the vertical GRF in the current study is about 0.3 BW. Our results agree with other studies which show that the feet support about 20% to 30% BW during sitting pivot transfers (Gagnon, Nadeau, Noreau, Dehail, & Gravel, 2008). There was a tendency to see the highest VGRF and FFM for the HH-A technique, most likely because this technique facilitates forward trunk flexion transferring more body weight through the legs than the arms. It may be important to consider trade-offs when teaching transfer techniques. A technique that off-loads shoulder loading such as the Head-Hips techniques (Koontz, Kankipati, Lin, Cooper, & Boninger, 2011b) may in turn increase loads through the legs predisposing the individual with weaker limbs to fractures.
The HGRF, or transverse loading, may cause breaks in the third metatarsal bones of runners (Arangio, Beam, Kowalczyk, & Salathe, 1998). The HGRF in this study was lower than that found during running. The VLR was also lower than for studies on running and fracture risk likely due to slower speeds of movement and less weight on the lower limbs. (Arangio, et al., 1998; Dixon, Creaby, & Allsopp, 2006; Zadpoor & Nikooyan, 2011) . Stabilizing the feet, using appropriate handgrips, and executing control over the flight and landing is recommended for safe transfers (McClure, et al., 2011). To avoid rapid or impulse loading of force and torque on the lower extremity, the quality of transfer should be emphasized over speed. Because transfer FFM is very similar to the published values linked to stress-fractures in able-bodied activities and may be largely unmodifiable for sitting-pivot transfers, it’s critical to keep control over the rate of loading which more likely can be modified with transfer training and upper limb conditioning.
The experimental setup may have influenced the subjects’ normal transfer pattern. For example, both feet had to be positioned and stabilized on the force plate throughout the transfer process. Thus, subjects who typically use only one foot down or keep both their feet in the foot rest could and likely do experience higher levels of loading than what was observed in this study. In the future, we will combine kinetic and kinematic data to analyze ankle and knee joint biomechanics during transfers and investigate the impact of wheelchair positioning on upper and lower limb kinetics. This study is the first step towards determining best transfer movement patterns and positioning to prevent lower extremity fractures.
Study limitations and future work
The experimental setup may have influenced the subjects’ normal transfer pattern. For example, both feet had to be positioned and stabilized on the force plate throughout the transfer process. Thus, subjects who typically use only one foot down or keep both their feet in the foot rest could and likely do experience higher levels of loading than what was observed in this study. A majority of the subjects in this study (73%) had an incomplete SCI and some level of voluntary motor function in the lower extremities. It’s possible they may be generating more or able to endure more FFM and GRF than persons without leg function. A future study with a larger sample is needed in order to study more conclusively the effects of leg function on the FFM and GRF during transfers.
In the future, we will combine kinetic and kinematic data to analyze ankle and knee joint biomechanics during transfers and investigate the impact of wheelchair positioning on upper and lower limb kinetics. This study is the first step towards determining best transfer movement patterns and positioning to prevent lower extremity fractures.
REFERENCES
- Arangio, G. A., Beam, H., Kowalczyk, G., & Salathe, E. P. (1998). Analysis of stress in the metatarsals. Foot and Ankle Surgery 4, 123-128.
- Caulfield, B., & Garrett, M. (2004). Changes in ground reaction force during jump landing in subjects with functional instability of the ankle joint. Clin Biomech (Bristol, Avon), 19(6), 617-621.
- Chantraine, A., Nusgens, B., & Lapiere, C. M. (1986). Bone remodeling during the development of osteoporosis in paraplegia. Calcif Tissue Int, 38(6), 323-327.
- Dixon, S. J., Creaby, M. W., & Allsopp, A. J. (2006). Comparison of static and dynamic biomechanical measures in military recruits with and without a history of third metatarsal stress fracture. Clin Biomech (Bristol, Avon), 21(4), 412-419.
- Fattal, C., Mariano-Goulart, D., Thomas, E., Rouays-Mabit, H., Verollet, C., & Maimoun, L. (2011). Osteoporosis in persons with spinal cord injury: the need for a targeted therapeutic education. Arch Phys Med Rehabil, 92(1), 59-67.
- Finley, M. A., McQuade, K. J., & Rodgers, M. M. (2005). Scapular kinematics during transfers in manual wheelchair users with and without shoulder impingement. Clin Biomech (Bristol, Avon), 20(1), 32-40.
- Gagnon, D., Nadeau, S., Noreau, L., Dehail, P., & Gravel, D. (2008). Quantification of reaction forces during sitting pivot transfers performed by individuals with spinal cord injury. J Rehabil Med, 40(6), 468-476.
- Gagnon, D., Nadeau, S., Noreau, L., Dehail, P., & Piotte, F. (2008). Comparison of peak shoulder and elbow mechanical loads during weight-relief lifts and sitting pivot transfers among manual wheelchair users with spinal cord injury. J Rehabil Res Dev, 45(6), 863-873.
- Gagnon, D., Nadeau, S., Noreau, L., Eng, J. J., & Gravel, D. (2008). Trunk and upper extremity kinematics during sitting pivot transfers performed by individuals with spinal cord injury. Clin Biomech (Bristol, Avon), 23(3), 279-290.
- Gottschall, J. S., & Kram, R. (2005). Ground reaction forces during downhill and uphill running. J Biomech, 38(3), 445-452.
- Kankipati, P., Koontz, A. M., Vega, A., & Lin, Y. S. (2011). Phase Identification of Sitting Pivot Wheelchair Transfers. Paper presented at the American Society of Biomechanics.
- Kiss, R. M. (2010). Comparison between kinematic and ground reaction force techniques for determining gait events during treadmill walking at different walking speeds. Med Eng Phys, 32(6), 662-667.
- Koontz, A. M., Kankipati, P., Lin, Y. S., Cooper, R. A., & Boninger, M. L. (2011a). Upper limb kinetic analysis of three sitting pivot wheelchair transfer techniques. Clin Biomech (Bristol, Avon), 26(9), 923-929.
- Koontz, A. M., Kankipati, P., Lin, Y. S., Cooper, R. A., & Boninger, M. L. (2011b). Upper limb kinetic analysis of three sitting pivot wheelchair transfer techniques. Clin Biomech (Bristol, Avon).
- Koontz, A. M., Lin, Y. S., Kankipati, P., Boninger, M. L., & Cooper, R. A. (2011). Development of custom measurement system for biomechanical evaluation of independent wheelchair transfers Journal of Rehabilitation Research & Development, 48(8), 1015- 1028.
- Lazo, M. G., Shirazi, P., Sam, M., Giobbie-Hurder, A., Blacconiere, M. J., & Muppidi, M. (2001). Osteoporosis and risk of fracture in men with spinal cord injury. Spinal Cord, 39(4), 208-214.
- McClure, L. A., Boninger, M. L., Ozawa, H., & Koontz, A. (2011). Reliability and validity analysis of the transfer assessment instrument. Arch Phys Med Rehabil, 92(3), 499-508.
- Milner, C. E., Davis, I. S., & Hamill, J. (2006). Free moment as a predictor of tibial stress fracture in distance runners. J Biomech, 39(15), 2819-2825.
- Patatoukas, D., Farmakides, A., Aggeli, V., Fotaki, S., Tsibidakis, H., Mavrogenis, A. F., et al. (2011). Disability- related injuries in athletes with disabilities. Folia Med (Plovdiv), 53(1), 40-46.
- Pohl, M. B., Mullineaux, D. R., Milner, C. E., Hamill, J., & Davis, I. S. (2008). Biomechanical predictors of retrospective tibial stress fractures in runners. J Biomech, 41(6), 1160-1165.
- Preservation of upper limb function following spinal cord injury: a clinical practice guideline for health-care professionals. (2005). J Spinal Cord Med, 28(5), 434- 470.
- Saverino, A., Benevolo, E., Ottonello, M., Zsirai, E., & Sessarego, P. (2006). Falls in a rehabilitation setting: functional independence and fall risk. Eura Medicophys, 42(3), 179-184.
- Szollar, S. M., Martin, E. M., Sartoris, D. J., Parthemore, J. G., & Deftos, L. J. (1998). Bone mineral density and indexes of bone metabolism in spinal cord injury. Am J Phys Med Rehabil, 77(1), 28-35.
- Vestergaard, P., Krogh, K., Rejnmark, L., & Mosekilde, L. (1998). Fracture rates and risk factors for fractures in patients with spinal cord injury. Spinal Cord, 36(11), 790- 796.
- Wilmet, E., Ismail, A. A., Heilporn, A., Welraeds, D., & Bergmann, P. (1995). Longitudinal study of the bone mineral content and of soft tissue composition after spinal cord section. Paraplegia, 33(11), 674-677.
- Zadpoor, A. A., & Nikooyan, A. A. (2011). The relationship between lower-extremity stress fractures and the ground reaction force: a systematic review. Clin Biomech (Bristol, Avon), 26(1), 23-28.
Acknowledgements
This material is based upon work supported by the Department of Veterans Affairs (A4489R) and the National Science Foundation, Project EEC 0552351.
The contents of this paper do not represent the views of the Department of Veterans Affairs or the United States Government.