Sharon Stansfield, Nancy Rader, Carole Dennis, Judith Pena-Shaff and Hélène Larin
Ithaca College
INTRODUCTION
Executive functions (EFs) include mental processes such as inhibition and interference control, working memory, and cognitive flexibility, that are important for cognitive and social development [1]. Research indicates that the onset of self-locomotion (crawling on hands and knees) contributes to the development of executive function in infants [2]. Kermoian and Campos [3] found that 8.5 month old infants who either crawled on hands and knees or used a walker performed better on the object permanence test, a measure of EF, than did non-locomotor infants of the same age. Likewise, Bell and Fox [4] studied pre-crawling infants and infants who had experience with hands and knees crawling at 8 months of age and found that crawling infants performed better on the object permanence test; they also noted locomotor group differences in brain electrical activity at lateral frontal and parietal sites. Piek, [5] evaluated the fine and gross motor performance of each of 51 infants at 4, 6, 8, 12, 16, 18, 20, 24, 30, 36, and 48 months of age. They then measured the cognitive development of 33 of these children at school age (6-11 years old) and found a significant relationship between gross motor ability as an infant and the cognitive indices of working memory and processing speed at school age.
Most previous studies comparing the development of executive function in locomotor and non-locomotor children have been correlational, meaning that there is a relationship between locomotor experience and development of EF, but that no clear cause and effect can be established. In this paper we present the results of an experimental study on the effect of robot-assisted self-locomotion on EF (specifically, object permanence using the A-not-B task) in pre-crawling infants. The hypothesis was that there would be a causal effect between infants with self-locomotor experience and superior performance on the A-not-B object permanence task.
METHODS
Participants
Participants were 61 typically developing, pre-crawling, 5-month old infants who were randomly assigned to either a robot-assisted locomotor group or a non-locomotor, control group.
Procedure
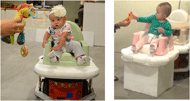
The A-not-B task
The A-not-B object permanence task was administered to all infants in the study after they completed the twelve sessions and before they reached age 7.5 months. In an A-not-B task, infants learn to find an object at location A then must inhibit that response in order to learn to find the object when it moves to location B. The A-not-B task is considered a measure of EF as it requires an understanding of object permanence / working memory, as well as searching and planning. A typical A-not-B task either requires infants to reach toward and remove a cover to find the object in either location A or location B, or records their looking behavior relative to the A or B location. Cuevas and Bell [7] found that infants perform better on the looking version from 5-7 months and then equally well on either version from 8-10 months, in part due to the continued development of reaching skills. In order to reduce reliance on motor development, this study examined looking behavior of infants in a digital A-not-B task using an eye gaze tracker and an interactive A-not-B simulation that was programmed using the Unity game engine.
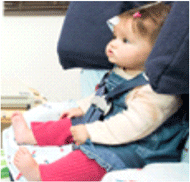
MEASURES
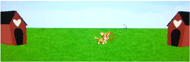
The A-not-B simulation has four phases, each containing six trials. During each trial of each phase, a cartoon dog is shown in the middle of the screen and a barking sound is played. The dog then runs into a doghouse and disappears from the screen. Between each trial, a green screen is shown for 1 second prior to showing the dog again in the center of the screen. During phase 0, the training phase, the dog barks and runs to a doghouse on the left side of the screen and immediately comes out of the doghouse and barks again. In phase 1, the dog does not come out and bark unless the child looks at the doghouse within 1 second (A condition). Phase 2 shows a second doghouse on the right side of the screen, but the dog still runs to the left doghouse (A condition with distractor). Phase 3 has the dog running to the right doghouse (B condition), again not coming out unless the infant looks at the doghouse within 1 second of the dog entering. Phase 4 is the same as phase 3, but with a four second green screen delay between trials. Figure 3 shows an image from the A-not-B simulation.
Valid data for 26 infants (13 control and 13 experimental) was used. Other data were eliminated due to various factors such as problems with data capture, the infant being fussy or never looking at the screen, etc. This loss of data is typical for infant research. The data for these 26 infants was coded for each phase according to the number of times the infant looked toward the correct doghouse (the one the dog had entered) in anticipation of the dog emerging. Infants received 1 point if they looked at the correct doghouse in 4 or more trials during a six-trial phase. These points were totaled for an A-not-B score with a possible maximum of four points (1 for each of the four phases).
RESULTS
An Independent Samples t-test indicated a statistically significant difference between the A-not-B scores for the locomotor group (M = 2.15, SD = .80) and the non-locomotor group (M = 1.46, SD = .66), t(26) = 2.41, p = .012, effect size = .194. Univariate Analysis of Variance tests of between-subjects effects found no significant difference for gender (p = .354), but a significant difference was found for condition (p = .024.). As hypothesized, infants in the locomotor group made more anticipatory looks at the correct doghouse than did the infants in the non-locomotor group.
DISCUSSION
Previous correlational studies have shown a relationship between an infant gaining self-locomotion and development of EF [8], [9]. The results of the experimental study presented here demonstrate a causal relationship between the two. Pre-crawling, typically developing infants provided with the ability to self-locomote showed better performance on the A-not-B task than did infants in the control group. This finding, in addition to its contribution to developmental science, also has implications for the cognitive development of infants with motor impairment.
Indeed, a substantial body of research indicates that infants who have experience with self-produced locomotion may possess developmental attributes that are not shared by infants lacking this experience due to motor impairment. Specifically, executive dysfunction has been associated with a number of conditions that involve motor impairment, including Down syndrome [10], developmental coordination disorder (Wilson, Ruddock, et al., 2013), autism [12], cerebral palsy [13], spina bifida [14], hypotonia [15] as well as Williams syndrome and very pre-term birth [16]. Although many of these conditions are associated with neurological impairments, evidence is growing to support the view that mobility impairment may indeed contribute to cognitive dysfunction [2]. If locomotor impairment does lead to secondary deficits in executive functioning, it would be important to provide self-locomotion to infants with mobility impairment. This study furthers the hypothesis that there is a causal link between locomotion and executive function and supports arguments for providing mobility devices to children with motor impairment as early as possible [17]–[21].
REFERENCES
[1] A. Diamond, “Executive Functions,” Annu. Rev. Psychol., vol. 64, pp. 135–168, 2013.
[2] D. I. Anderson et al., “The role of locomotion in psychological development,” Front. Psychol., vol. 4, no. JUL, pp. 1–17, 2013.
[3] R. Kermoian and J. J. Campos, “Locomotor experience: a facilitator of spatial cognitive development.,” Child Dev., vol. 59, no. 4, pp. 908–17, Aug. 1988.
[4] M. A. Bell and N. A. Fox, “Crawling experience is related to changes in cortical organization during infancy: evidence from EEG coherence.,” Dev. Psychobiol., vol. 29, no. 7, pp. 551–561, Nov. 1996.
[5] J. P. Piek, L. Dawson, L. M. Smith, and N. Gasson, “The role of early fine and gross motor development on later motor and cognitive ability,” Hum. Mov. Sci., vol. 27, pp. 668–681, 2008.
[6] S. Stansfield, C. Dennis, and H. Larin, “WeeBot: A novel method for infant control of a robotic mobility device,” in Proceedings - IEEE International Conference on Robotics and Automation, 2012.
[7] K. Cuevas and M. A. Bell, “Developmental Progression of Looking and Reaching Performance on the A-not-B Task,” Dev. Psychol., vol. 46, no. 5, pp. 1363–1371, 2010.
[8] J. J. Campos, D. I. Anderson, M. A. Barbu-Roth, E. M. Hubbard, M. J. Hertenstein, and D. Witherington, “Travel broadens the mind,” Infancy, vol. 1, no. 2, pp. 149–219, 2000.
[9] D. H. Rakison and A. L. Woodward, “New Perspectives on the Effects of Action on Perceptual and Cognitive Development,” Dev. Psychol., vol. 44, no. 5, pp. 1209–1213, 2008.
[10] N. Schott and B. Holfelder, “Relationship between motor skill competency and executive function in children with Down’s syndrome,” J. Intellect. Disabil. Res., vol. 59, no. 9, pp. 860–872, 2015.
[11] P. H. Wilson, S. Ruddock, B. Smits-Engelsman, H. Polatajko, and R. Blank, “Understanding performance deficits in developmental coordination disorder: A meta-analysis of recent research,” Dev. Med. Child Neurol., vol. 55, no. 3, pp. 217–228, 2013.
[12] E. L. Hill, “Executive dysfunction in autism,” Trends Cogn. Sci., vol. 8, no. 1, pp. 26–32, 2004.
[13] L. Weierink, R. J. Vermeulen, and R. N. Boyd, “Brain structure and executive functions in children with cerebral palsy: A systematic review,” Res. Dev. Disabil., vol. 34, no. 5, pp. 1678–1688, 2013.
[14] H. B. Taylor, S. H. Landry, M. Barnes, P. Swank, L. B. Cohen, and J. Fletcher, “Early information processing among infants with and without spina bifida,” Infant Behav. Dev., vol. 33, no. 4, pp. 365–372, 2010.
[15] L. F. Koziol and L. A. Barker, “Hypotonia, Jaundice, and Chiari Malformations: Relationships to Executive Functions,” Appl. Neuropsychol. Child, vol. 2, pp. 141–149, 2013.
[16] J. Atkinson and O. Braddick, “From genes to brain development to phenotypic behavior: ‘Doral – stream vulnerability’ in relation to spatial cognition, attention, and planning of actions,” in Progress in Brain Research: Vol. 189, Gene expression to neurobiology and behavior: Human brain development and developmental disorders, O. Braddick, J. Atkinson, and G. Innocenti, Eds. Elsevier, 2011, pp. 261–283.
[17] C. Butler, “Effects of powered mobility on self-initiated behaviors of very young children with locomotor disability,” Dev. Med. Child Neurol., vol. 28, no. 3, pp. 325–332, 1986.
[18] M. A. Jones, I. R. McEwen, and B. R. Neas, “Effects of power wheelchairs on the development and function of young children with severe motor impairments.,” Pediatr. Phys. Ther., vol. 24, no. 2, p. 131–40; discussion 140, Jan. 2012.
[19] P. Guerette, J. Furumasu, and D. Tefft, “The Positive Effects of Early Powered Mobility on Children’s Psychosocial and Play Skills,” Assist. Technol., vol. 25, no. 1, pp. 39–48, 2013.
[20] S. W. Logan, H. A. Feldner, J. C. Galloway, and H.-H. Huang, “Modified Ride-on Car Use by Children With Complex Medical Needs,” Pediatr. Phys. Ther., vol. 28, no. 1, pp. 100–107, 2016.
[21] L. K. Kenyon, J. P. Farris, K. Gallagher, L. Hammond, L. M. Webster, and N. J. Aldrich, “Power mobility training for young children with multiple, severe impairments: A case series.,” Phys. Occup. Ther. Pediatr., vol. 37, no. 1, pp. 19–34, 2017.
ACKNOWLEDGEMENTS
This work was supported by the National Science Foundation under grant number PD 08-1698 BCS Developmental Learning Sciences/CRI. The analysis would not have been possible without the efforts of Emma Enav and Alexa Lesley who coded all of the eye gaze data.