Shriniwas Patwardhan, Susannah Engdahl, Biswarup Mukherjee, Ahmed Bashatah, Ananya Dhawan, Rushna Abreu, and Siddhartha Sikdar
Department of Bioengineering, George Mason University
INTRODUCTION
There are currently around 41,000 individuals in the United States living with major upper limb amputation, and this number is expected to double by 2050 [1]. Many individuals with upper limb amputation reject their prostheses due to dissatisfaction with the devices [2-3]. Most existing myoelectric prostheses use dual-site surface electromyography (sEMG) to implement direct control of a terminal device. Achieving reliable proportional position control with sEMG is difficult due to random fluctuations in the sEMG signal leading to low signal to noise ratio [4–7]. As a result, the sEMG signal is typically thresholded to drive joint velocity, rather than position. One way to implement velocity control is with a fixed velocity paradigm, in which the user activates the flexor or extensor muscles to initiate opening or closing of the terminal device and relaxes to stop the terminal device. Thus, the user achieves a target position of the terminal device by maintaining flexion or extension for a given period of time. This strategy can make it challenging to achieve a desired target position, e.g., to rotate the wrist to a desired angle.
Prior work from our laboratory has shown that real-time ultrasound imaging of forearm muscle deformations during volitional motor activity can be used for real-time classification of multiple degrees of freedom in able-bodied individuals [8,9] and individuals with transradial amputation [8]. This method, called sonomyography, offers a reliable way to measure muscle deformation, as opposed to electrical signals from deep musculature. Some of the problems associated with sEMG control are overcome by using sonomyography, since it gives direct access to muscle movements deep below the skin. Sonomyography has improved signal to noise ratio compared to sEMG, thereby enabling the possibility of direct position control. Recently, we have proposed a proportional position control paradigm using ultrasound sensing [10], and shown feasibility in able-bodied subjects and prosthetic users. In position control, users are able to directly achieve a target position by controlling the extent of muscle deformation.
The aim of this study is to compare the position and velocity control modalities to determine which would be more efficient to use in the development of future prosthetic devices. In this work, we compared between velocity control and position control paradigms for a virtual cursor control task using sonomyography. We hypothesized that compared to velocity control, more targets would be acquired in position control, and the time taken to acquire those targets would be reduced.
METHODS
A total of ten naive, able-bodied individuals (mean age: 29 4 years; five male) were recruited and provided written informed consent for this study. Nine of the participants reported being right-handed. All experiments in this work were approved by the George Mason University Institutional Review Board.
Participants were asked to sit upright with their elbow below their shoulder and the forearm comfortably secured to a platform on the armrest of the chair, which was positioned at a fixed distance from a computer monitor. Participants were instrumented with a clinical ultrasound system (Terason uSmart 3200T) connected to a low-profile, high frequency, linear, 16HL7 transducer. The imaging depth was set to 4 cm and the gain was set to 60. The transducer was manually positioned on the volar aspect of the dominant forearm in order to access the deep and superficial flexor muscles of the forearm. The transducer was secured in a custom designed probe holder and held in place with a stretchable cuff. In order to ensure that participants were relying on their proprioceptive sense to perform each task, they placed their hand in an opaque enclosure to prevent direct observation of the wrist and hand movements. A USB-based video grabber (DVI2USB 3.0, Epiphan Systems, Inc.) was used to transfer ultrasound image sequences in real time to a PC (Dell XPS 15 9560). The acquired image frames were processed in MATLAB (The MathWorks, Inc.) using custom-developed algorithms that we have described previously elsewhere [10]. Following probe placement, the participants were randomly selected to perform either the position or velocity control task first. They were given a one-minute break and then tested on the second technique.
Position control:
Prior to position control testing, participants underwent a training procedure in which they performed repeated wrist extension and flexion. These movements were timed to a metronome such that the participant was cued to transition from full extension to full flexion within one second, hold the position for one second, return to full extension within one second, and hold the position for one second. This process was repeated five times. The ultrasound images corresponding with the full extension and flexion phases were averaged into a single representative image and added to a training database with a corresponding "extension" or "flexion" label.
During the position control task, the participant controlled an on-screen cursor that could move horizontally in proportion to the degree of muscle activation in the forearm (i.e., the cursor moved left in response to wrist flexion and right in response to wrist extension for right-handed subjects and opposite for left-handed subjects). The objective was to reach a vertical target line as quickly as possible and retain the cursor at the target line until the next target was presented. The interface presented the target position at random from a set of nine predefined, equidistant positions. The target remained at each position for 10 seconds and then moved the next position until all nine target points were presented. For each target position, the participant was prompted, via an automated audio cue, to move the cursor to the extend position before the task began. After the nine targets were presented, the participant would rest for one minute and then repeat the task. They completed a six-task block, with the first block for practice, and rest five blocks were used for analysis.
Velocity control:
Prior to velocity control testing, participants underwent a training procedure that was similar to the one used for position control. Participants transitioned between rest and full wrist extension for a total of five times, then repeated the procedure to transition between rest and full wrist flexion instead. Thus, the training database contained ultrasound images for "rest", "extension", and "flexion."
The objective of the velocity control task was identical to that of the position control task. The only difference was that the cursor moved horizontally at a fixed velocity in response to muscle activity in the forearm (i.e., the cursor moved left in response to wrist flexion, right in response to wrist extension, and remained stationary when the wrist was in a neutral position for right-handed subjects). A fixed velocity of 79.4% (of the full range of target positions) per second was selected based on an average of commercially available prosthetic hands [11].
Data analysis:
During post-hoc analysis, the trials were marked as completed if the user held the cursor inside the target bound for a stipulated amount of time. There were no bounds imposed during data acquisition, but a number of different possible target bounds were imposed post-hoc. We quantified the target acquisition rate and time needed to reach the target under a range of bound sizes (5% to
15% of the target) and imposed hold times (1 to 3 seconds).
RESULTS
A complete data set was available for analysis from only nine of the participants due to a technical error during data collection.
At the small target thickness of ± 5%, subjects completed 82% more trials in position control (87.58 ± 3.44%) as opposed to velocity control (48.10 ± 2.38%) across all hold times (Figure 1). As the target thickness was increased, the difference between the two control modalities decreased. At the largest target thickness of ± 15%, subjects completed 12% more trials in position control (96.08 ± 0.66%) as opposed to velocity control (78.91 ± 5.62%) across all hold times. Velocity control never outperformed position control in terms of target completion rate. A paired t-test revealed that the target completion rate for position control was significantly higher than for velocity control across all hold times and target thicknesses (p < 0.05).
At the small target thickness of ± 5%, subjects acquired the target faster using velocity control (3.15 ± 0.93 seconds) as opposed to position control (4.69 ± 1.26 seconds), across all target locations. At the largest target thickness of ± 15%, subjects acquired the target faster using velocity control (2.52 ± 1.10 seconds) as opposed to position control (3.16± 1.34 seconds) across all target locations. A paired t-test showed that the time to target for position control was significantly higher than that for velocity control across all target locations and thicknesses except one target location (50%) and target thickness (15%) (p < 0.05).
DISCUSSION
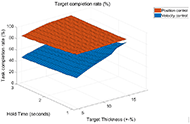
In the current experiment, we implemented a virtual target acquisition task in which the cursor position was manipulated using sonomyographic control. We tested for the effect of hold time, target location, and target thickness on the target completion rate and the time taken to acquire the target. Our main finding is that for all investigated values of target thickness and hold time, position control had a higher target completion rate as compared to velocity control, while it also had a higher time to target for nearly all values of target location and target thickness.
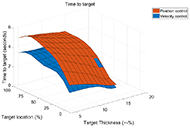
This finding suggests that in the context of prosthesis control, it may be faster to attain a certain hand pose using velocity control, but position control may be preferred where fine manipulation is required to reach a specific target. During velocity control, a user may just maintain a maximal flexion of their muscles so the hand moves towards the given pose as fast as possible, so the position may be acquired quickly. Once they are close to the required hand pose, it is very hard to acquire it exactly. During position control, the user can manipulate the position of the prosthetic hand more finely since the position correlates with the intensity of the biosignal used for control. While our experiment evaluated only target position, we believe the results would also hold for grip force, which will be evaluated in a future study.
To the best of our knowledge, this is the first study using ultrasound as the signal to compare position and velocity control. Previous studies have used a force sensor to compare between them, and found similar results. Our results are partially in contradiction of their results. We found that the target completion rate was higher whereas the time to target was worse for position control as opposed to velocity control. In terms of maximum overshoot, our results are similar.
This study was limited by a small sample size of 9 subjects. The fixed velocity used in this experiment was based on a survey of the literature [11]. We took the average closing time for the prosthetic hands whose specifications were given in the literature, and used that as the time taken to move the cursor from one end of the screen to another. In future work, we will implement a variable velocity approach, to make the experiment as analogous to a real-world prosthetic hand as possible.
CONCLUSION
Traditional myoelectric prosthesis direct control systems utilize velocity control to achieve target position of a terminal device. Sonomyography, an emerging biosignal sensing method that has improved signal to noise ratio compared to electromyography, can enable robust proportional positional control. In this study, we utilized sonomyography to investigate the advantages of position and velocity control using a virtual cursor control task. Position control outperformed velocity control in terms of target completion rate for all the investigated values of target thickness, target location, and hold time. At the same time, velocity control outperformed position control in terms of time to target for all but one of the values investigated values of target thickness (15%) and target location (50%).
REFERENCES
- Ziegler-Graham K, MacKenzie EJ, Ephraim PL, Travison TG, Brookmeyer R. Estimating the Prevalence of Limb Loss in the United States: 2005 to 2050. Arch Phys Med Rehabil. 2008 Mar;89(3):422–9.
- Biddiss E, Beaton D, Chau T. Consumer design priorities for upper limb prosthetics. Disabil Rehabil Assist Technol. 2007;2(6):346–57.
- Wright TW, Hagen AD, Wood MB. Prosthetic usage in major upper extremity amputations. J Hand Surg. 1995;20(4):619–22.
- Clancy EA, Morin EL, Merletti R. Sampling, noise-reduction and amplitude estimation issues in surface electromyography. J Electromyogr Kinesiol. 2002;12(1):1–16.
- Fillauer CE, Pritham CH, Fillauer KD. Evolution and development of the silicone suction socket (3S) for below-knee prostheses. J Prosthet Orthot. 1989;1(2):92–103.
- Kong YK, Hallbeck MS, Jung MC. Crosstalk effect on surface electromyogram of the forearm flexors during a static grip task. J Electromyogr Kinesiol. 2010;20(6):1223–9.
- van Duinen H, Gandevia SC, Taylor JL. Voluntary activation of the different compartments of the flexor digitorum profundus. J Neurophysiol. 2010;104(6):3213–21.
- Akhlaghi N, Baker CA, Lahlou M, Zafar H, Murthy KG, Rangwala HS, et al. Real-Time Classification of Hand Motions Using Ultrasound Imaging of Forearm Muscles. IEEE Trans Biomed Eng. 2016 Aug;63(8):1687–98.
- Sikdar S, Rangwala H, Eastlake EB, Hunt IA, Nelson AJ, Devanathan J, et al. Novel Method for Predicting Dexterous Individual Finger Movements by Imaging Muscle Activity Using a Wearable Ultrasonic System. IEEE Trans Neural Syst Rehabil Eng. 2014 Jan;22(1):69–76.
- Dhawan AS, Mukherjee B, Patwardhan S, Akhlaghi N, Levay G, Holley R, et al. Proprioceptive Sonomyographic Control: A novel method of intuitive proportional control of multiple degrees of freedom for upper-extremity amputees. ArXiv180806543 Cs [Internet]. 2018 Aug 20 [cited 2019 Jun 13]; Available from: http://arxiv.org/abs/1808.06543
- Belter JT, Segil JL, Dollar AM, Weir RF. Mechanical design and performance specifications of anthropomorphic prosthetic hands: a review. J Rehabil Res Dev. 2013;50(5):599–618.